The Large Hadron Collider is known for discovering the Higgs boson, but the technologies used in accelerating and detecting new particles also have uses outside of high-energy physics. Carsten Welsch explains how technology transfer is creating a new generation of compact accelerators with applications ranging from security to cancer treatment
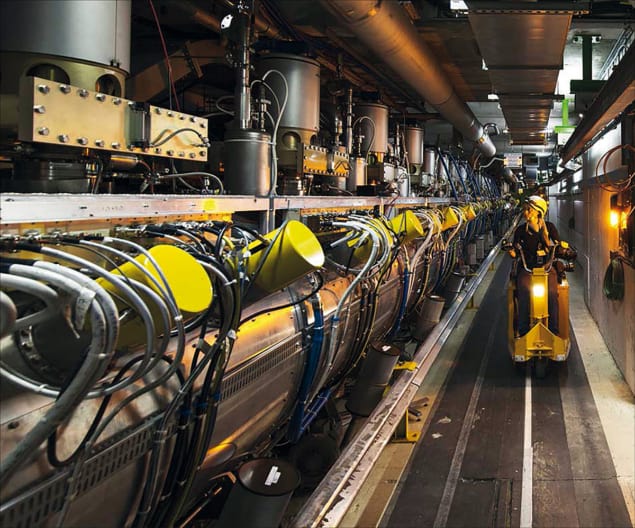
Passengers at London’s Heathrow Airport got some good news recently when it was announced that – thanks to the airport’s new computerized tomography (CT) scanners – they will soon be able to stop separating out the liquids and gels in their hand luggage as they go through security. The new scanners produce high-resolution, three-dimensional X-ray images in real time, making it easier to detect explosives quickly, without the need for a separate screening process.
This development was widely reported in the media and heralded as a boon for travellers and security staff alike. What was not so widely reported, however, is that streamlined imaging of luggage and containers has been achieved, in part, by improvements to the accelerators that provide the electron beams for the scanners. These improvements have made scanning equipment more compact, while also enhancing the quality of the imaging beam – and they were based on knowledge that has been acquired, directly and indirectly, from R&D into particle accelerators used for scientific research.
Real-time security screening
In CT scanning – still the most widely-used imaging technique for luggage – a beam of particles (traditionally X-rays, but it could also be neutrons or other particles) is sent through the object. By measuring the attenuated beam, and then repeating this process in steps over a 180- or 360 ˚ range, the imaging system produces a 2D shadowgraph of the specimen, revealing details of its internal structure (dimensions, shape, internal defects, density, and so on). These cross-sectional images are reconstructed using projections from several directions and visualized using 3D graphics-rendering software. The 3D image is then manipulated and sliced in various ways to provide a thorough understanding of the object.
Although many reconstruction algorithms exist, most fall into one of two categories: filtered back projection (FBP) and iterative reconstruction (IR). Both procedures give inexact results and there is a trade-off between accuracy and computation time required. FBP demands less computing power but is less accurate, while IR generally produces fewer artefacts (errors in the reconstruction) at a higher computing cost. In both cases, the computing power requirement means that most tomographic scanners acquire static images, not moving ones. Meanwhile, the large size and substantial cost of the accelerators used to create the particle beams ensures that although CT is routinely used to scan hold luggage, its wider use in security screening is limited.
Recently, however, real-time tomographic (RTT) scanners have emerged that significantly reduce these obstacles. The first models were relatively slow, as they were mounted on a gantry and the scanner was moved around the bag, typically taking 12 to 15 views. However, the new generation of RTT systems have no moving parts and are considerably faster.
The manufacturer of Heathrow’s £50m CT scanners has not been disclosed, but one of the most advanced systems is made by a US-based company, Rapiscan, and was developed with support and training from scientists at the UK’s Daresbury Laboratory (operated by the Science and Technology Facilities Council). The Rapiscan baggage scanning system uses multiple X-ray sources. A stationary array of micro X-ray emitters captures tens of thousands of views of a bag, generating images with significantly better resolution in all planes than standard CT imaging. A very fast reconstruction algorithm has also been developed to speed up the screening process, making it possible to check 1500 to 1800 bags per hour. This is hugely significant for the transport industry. Security checks at airports and docks constitute a major bottleneck in international travel and commerce, and unknown objects can cause long delays even when they turn out to be harmless.

Balancing quality with dosage
The manufacturers of particle accelerators used in security-screening equipment do not generally need to consider the radiation dose imparted to the suitcases, containers and so on being imaged. This is, of course, not true in medical imaging, where the dose to the patient is a vital consideration. In this field, CT scanning is widely used due to its high image quality, but recent research into the detrimental effects of higher radiation doses has drawn attention to an alternative technique known as digital tomosynthesis.
Digital tomosynthesis works by moving the X-ray source around the patient and acquiring images, as in computerized tomography. However, instead of a full 360 ˚ rotation, the angle is much smaller. This reduces the dose, but the smaller angle means that less information is available for the reconstruction, leading to lower image quality. Moving the source also creates motion-induced artefacts in the images.
An ongoing R&D effort by the accelerator science group at the University of Liverpool/Cockcroft Institute (which I lead) and a UK-based company, Adaptix, aims to design an ultra-compact, high-resolution digital tomosynthesis system that will offer enhanced medical imaging applications with less impact on the patient. The new designs include a system with multiple X-ray sources at different positions and angles that replace the one moving source. This minimizes motion-induced artefacts, although it also creates new challenges that we are still investigating. The hope is that the device will become an intermediate step between standard, low-dose planar X-rays and more expensive CT scanners, giving doctors the option of a tool that combines 3D imaging with a lower radiation dose.
Beating cancer sooner
A third example of technology transfer in accelerator science relates to cancer treatment. Proton- and ion-beam therapies are very effective at treating certain types of the disease, and they are a direct result from R&D into ion accelerators for fundamental science applications. Until recently, there was only one clinical facility using proton beams for cancer treatment in the UK: the Clatterbridge Cancer Centre on the Wirral, where 60 MeV protons have been used to treat ocular melanomas for more than 25 years. The first high-energy proton-beam cancer centre in the UK opened its doors in 2018 at the Christie hospital in Manchester, and more are currently being built.
Unlike the X-rays used in CT scanning and conventional radiotherapies, proton and ion beams used in cancer treatments do not pass all the way through the body. Instead, they stop sharply at a depth determined by their energy. By modulating the beam’s energy and direction, clinicians can deliver a homogeneous dose of radiation over a 3D tumour volume while sparing healthy surrounding tissue. In order to further optimize ion beam therapy, an international R&D effort has focused on the development of novel beam and patient imaging techniques, studies into enhanced biological and physical simulation models using Monte Carlo codes, and research into facility design and optimization to ensure optimum patient treatment along with maximum efficiency. These are all studies that build on research that originally targeted accelerators for fundamental science applications and show how health applications can benefit from more general R&D.
Accelerators that deliver high energies while maintaining a small physical “footprint” could bring research and applications that are currently only possible at large-scale facilities into wider use
Future benefits
These are just a few of the ways that R&D on particle accelerators has led to wider benefits. Other advances include better machine-learning techniques, robotics, new materials, and advances in cryogenics, data handling and analysis – and, of course, the World Wide Web, which was developed for particle physics experiments at CERN. These applications have changed our world, and I believe that future spin-out technologies will have just as much impact.
One reason for my optimism is that ongoing accelerator-science research promises technology innovations in a number of areas. The High Luminosity upgrade of the Large Hadron Collider (HL-LHC), for example, will make the world’s highest energy particle collider an even more powerful tool for discoveries.
Luminosity, originally defined to characterize the brightness of a star, determines how many collisions take place in a particle collider per unit of time. Currently, the beams in the LHC just cross over to create the opportunity for particles to collide; to maximize the chance of a smash, Cockcroft scientists have contributed to experiments with a method of capturing short bunches of protons a few centimetres long and throwing them sideways to hit another bunch of protons head-on. These “crab cavities” have the potential to increase the luminosity of the LHC by a factor of 10; this will speed up discovery to such a degree that 10 months of current work could be done in just one.
These developments will also demand novel beam diagnostics tools to fully characterize the more powerful beams, as existing technologies will simply no longer work. My group has been developing a non-invasive gas-jet-based monitor in close collaboration with experts from CERN and GSI in Germany for the LHC upgrade – a technology that shows great promise also for other high-energy and high-intensity accelerators.
Other strands of research aim to make accelerators more compact, thereby simplifying their operation and reducing their costs. International collaborations such as EuPRAXIA and AWAKE are investigating several mechanisms, including laser- and particle-beam-driven plasma acceleration. In these schemes, a plasma is modulated by a so-called “drive beam” that generates extremely high electric field gradients in the plasma. By injecting an electron beam into regions with a very high electric field, it has been shown that electrons can be accelerated to high energies over distances that are 1000 times shorter than is possible in conventional RF accelerators. These are very exciting developments, since accelerators that deliver high energies while maintaining a small physical “footprint” could bring research and applications that are currently only possible at large-scale facilities into wider use.
Further in the future, an even higher-energy collider (such as the Future Circular Collider being studied by researchers around the world) will require advances in several areas, including magnets, superconducting materials and cables, as well as detectors and diagnostics – all drivers of innovation that show great promise for application in other, often unexpected, areas. R&D into particle accelerators has been driving innovation for more than 100 years. This has resulted in applications with enormous benefits for society. An even more promising future lies ahead.